Table of Contents
- Introduction to Dielectric Materials
- Dielectric Properties
- Permittivity
- Dielectric Loss
- Dielectric Strength
- Dielectric Constant
- Types of Dielectric Materials
- Ceramic Dielectrics
- Polymer Dielectrics
- Glass Dielectrics
- Composite Dielectrics
- Applications of Dielectric Materials
- Capacitors
- Insulators
- Waveguides and Antennas
- Energy Storage Devices
- Factors Affecting Dielectric Properties
- Temperature
- Frequency
- Electric Field Strength
- Material Composition and Structure
- Measurement Techniques for Dielectric Properties
- Capacitance Measurement
- Impedance Spectroscopy
- Dielectric Relaxation Spectroscopy
- Dielectric Breakdown Testing
- Dielectric Materials in Emerging Technologies
- High-k Dielectrics for Microelectronics
- Dielectric Elastomers for Soft Robotics
- Dielectric Metamaterials
- Dielectric Materials for Energy Harvesting
- Challenges and Future Perspectives
- Frequently Asked Questions (FAQ)
- Conclusion
- References
Introduction to Dielectric Materials
Dielectric materials are electrical insulators that can be polarized by an applied electric field. They are characterized by their ability to store electrical energy in the form of an electric field, making them essential components in various electrical and electronic devices. Unlike conductors, dielectric materials have a high electrical resistance and low electrical conductivity, which allows them to prevent the flow of electric current while still being able to store and release electrical energy.
The unique properties of dielectric materials arise from their atomic and molecular structure. In dielectric materials, the electrons are tightly bound to their atoms, and the material does not contain free charge carriers like in conductors. When an external electric field is applied to a dielectric material, the electric field induces a displacement of the bound charges, leading to the formation of electric dipoles. This phenomenon is known as dielectric polarization.
Dielectric materials can be found in various forms, including solids, liquids, and gases. Some common examples of dielectric materials include ceramic, glass, plastic, rubber, and air. Each type of dielectric material has its own distinct properties and characteristics, making them suitable for different applications.
Understanding the fundamental properties of dielectric materials is crucial for designing and optimizing electrical and electronic devices. By carefully selecting and engineering dielectric materials, engineers and researchers can improve device performance, reduce power consumption, and enable new functionalities.
In the following sections, we will explore the key dielectric properties, types of dielectric materials, their applications, and the factors that influence their behavior. We will also discuss measurement techniques, emerging technologies, and future perspectives in the field of dielectric materials.
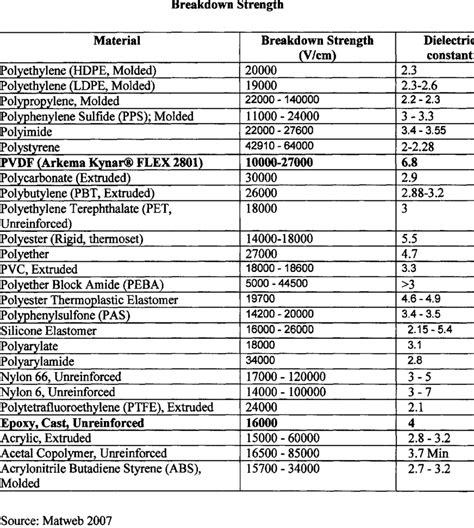
Dielectric Properties
Dielectric materials exhibit several unique properties that distinguish them from conductors and other types of materials. These properties are essential for understanding the behavior of dielectric materials and their suitability for various applications. In this section, we will discuss the four main dielectric properties: permittivity, dielectric loss, dielectric strength, and dielectric constant.
Permittivity
Permittivity is a measure of a dielectric material’s ability to store electrical energy in the form of an electric field. It is a complex quantity that consists of two components: the real part, known as the dielectric constant, and the imaginary part, known as the dielectric loss factor.
The real part of permittivity, denoted as ε’, represents the material’s ability to store electrical energy. It is related to the material’s polarizability, which describes how easily the material can be polarized by an external electric field. Materials with a high dielectric constant have a greater ability to store electrical energy compared to materials with a low dielectric constant.
The imaginary part of permittivity, denoted as ε”, represents the material’s ability to dissipate electrical energy in the form of heat. It is related to the material’s dielectric loss, which will be discussed in more detail in the next section.
Permittivity is typically expressed relative to the permittivity of free space, ε0, which has a value of approximately 8.85 × 10^-12 F/m. The relative permittivity, also known as the dielectric constant, is defined as the ratio of the material’s permittivity to the permittivity of free space:
εr = ε / ε0
where εr is the relative permittivity, ε is the material’s permittivity, and ε0 is the permittivity of free space.
Table 1 shows the relative permittivity values for some common dielectric materials:
Material | Relative Permittivity (εr) |
---|---|
Air | 1.0006 |
Teflon | 2.1 |
Paper | 3.5 |
Glass | 4-10 |
Mica | 5-8 |
Alumina | 9-10 |
Titanium dioxide | 80-100 |
Barium titanate | 1000-10000 |
As seen in Table 1, the relative permittivity values can vary widely depending on the material, ranging from close to 1 for air to over 1000 for some ceramics like barium titanate.
Dielectric Loss
Dielectric loss is a measure of a dielectric material’s ability to dissipate electrical energy in the form of heat. It is represented by the imaginary part of the complex permittivity, ε”, and is often expressed in terms of the loss tangent, tan δ, which is the ratio of the imaginary part to the real part of the permittivity:
tan δ = ε” / ε’
A high loss tangent indicates that the material has a high dielectric loss and will dissipate a significant amount of electrical energy as heat. Conversely, a low loss tangent indicates that the material has a low dielectric loss and will efficiently store electrical energy with minimal dissipation.
Dielectric loss is an important factor to consider when selecting materials for high-frequency applications, such as in telecommunications and radar systems. In these applications, materials with low dielectric loss are preferred to minimize signal attenuation and energy dissipation.
Table 2 shows the loss tangent values for some common dielectric materials at room temperature and a frequency of 1 MHz:
Material | Loss Tangent (tan δ) |
---|---|
Fused silica | 0.0001 |
Teflon | 0.0002 |
Polystyrene | 0.0002 |
Mica | 0.0003 |
Alumina | 0.001 |
Glass | 0.005-0.01 |
Nylon | 0.02-0.03 |
Bakelite | 0.05-0.1 |
As seen in Table 2, materials like fused silica, Teflon, and polystyrene have very low loss tangents, making them suitable for high-frequency applications. On the other hand, materials like nylon and Bakelite have higher loss tangents and are more suitable for low-frequency applications or situations where dielectric loss is less critical.
Dielectric Strength
Dielectric strength, also known as breakdown strength, is a measure of a dielectric material’s ability to withstand high electric fields without experiencing electrical breakdown. Electrical breakdown occurs when the applied electric field exceeds a critical value, causing the material to lose its insulating properties and become conductive.
The dielectric strength is typically expressed in terms of the maximum electric field that the material can withstand before breakdown occurs, and it is measured in units of volts per meter (V/m) or kilovolts per millimeter (kV/mm).
Materials with high dielectric strength are essential for applications that involve high voltages, such as high-voltage capacitors, power transmission equipment, and electrical insulation. A material with a higher dielectric strength can withstand higher voltages without failing, making it more suitable for these applications.
Table 3 shows the dielectric strength values for some common dielectric materials:
Material | Dielectric Strength (kV/mm) |
---|---|
Air (at 1 atm) | 3 |
Teflon | 60-173 |
Polyethylene | 18-50 |
Polypropylene | 30-40 |
Mica | 118-236 |
Glass | 30-550 |
Alumina | 13-16 |
Silicon dioxide | 470-670 |
As seen in Table 3, materials like Teflon, mica, glass, and silicon dioxide have high dielectric strengths, making them suitable for high-voltage applications. On the other hand, materials like air and polyethylene have lower dielectric strengths and are more suitable for low-voltage applications or situations where the electric field strength is not a critical factor.
It is important to note that the dielectric strength of a material can be influenced by various factors, such as temperature, humidity, impurities, and defects in the material. Therefore, the actual dielectric strength of a material in a specific application may differ from the values listed in Table 3, and it is essential to consider these factors when selecting materials for high-voltage applications.
Dielectric Constant
The dielectric constant, also known as the relative permittivity, is a dimensionless quantity that represents a material’s ability to store electrical energy in an electric field relative to that of vacuum. It is denoted by the symbol εr and is defined as the ratio of the material’s permittivity (ε) to the permittivity of free space (ε0):
εr = ε / ε0
where ε is the material’s permittivity, and ε0 is the permittivity of free space (approximately 8.85 × 10^-12 F/m).
A material with a higher dielectric constant can store more electrical energy in an electric field compared to a material with a lower dielectric constant. This property is essential for applications such as capacitors, where a high dielectric constant allows for the design of smaller, higher-capacity devices.
The dielectric constant is a function of the material’s polarizability, which describes how easily the material’s charges can be displaced by an external electric field. Materials with a higher polarizability will have a higher dielectric constant.
Table 4 shows the dielectric constant values for some common dielectric materials at room temperature:
Material | Dielectric Constant (εr) |
---|---|
Air | 1.0006 |
Teflon | 2.1 |
Polyethylene | 2.25 |
Polypropylene | 2.2-2.36 |
Paper | 3.5 |
Mica | 5-8 |
Glass | 4-10 |
Alumina | 9-10 |
Titanium dioxide | 80-100 |
Barium titanate | 1000-10000 |
As seen in Table 4, the dielectric constant values can vary widely depending on the material, ranging from close to 1 for air to over 1000 for some ceramics like barium titanate.
It is important to note that the dielectric constant of a material can be influenced by various factors, such as temperature, frequency, and electric field strength. Therefore, the actual dielectric constant of a material in a specific application may differ from the values listed in Table 4, and it is essential to consider these factors when selecting materials for applications that rely on the dielectric constant.
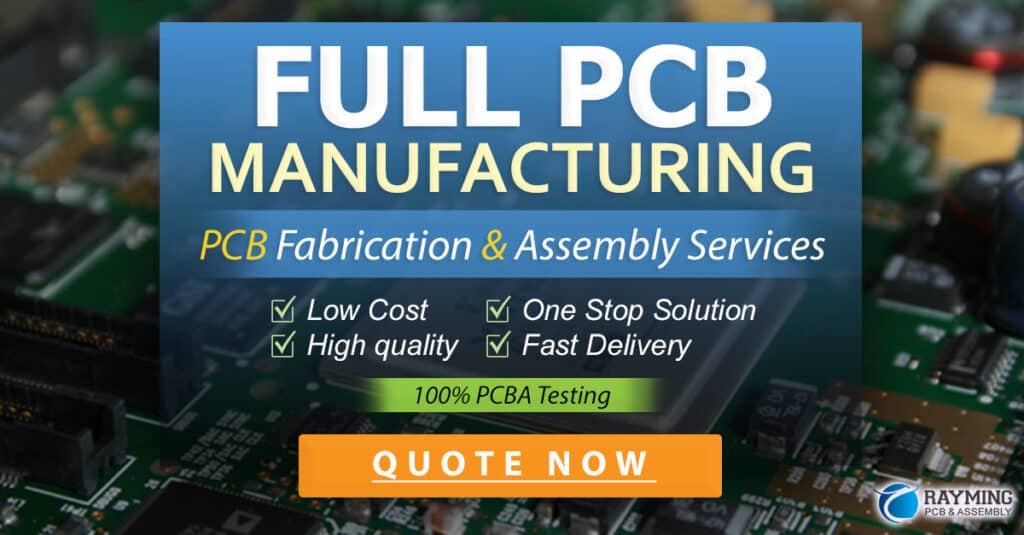
Types of Dielectric Materials
Dielectric materials can be classified into several categories based on their chemical composition, structure, and properties. Each type of dielectric material has its own unique set of characteristics that make it suitable for specific applications. In this section, we will discuss four main types of dielectric materials: ceramic dielectrics, polymer dielectrics, glass dielectrics, and composite dielectrics.
Ceramic Dielectrics
Ceramic dielectrics are inorganic, non-metallic materials that are composed of metal oxides, nitrides, or carbides. They are known for their high dielectric constant, low dielectric loss, and excellent thermal and chemical stability. Some common examples of ceramic dielectrics include alumina (Al2O3), titanium dioxide (TiO2), and barium titanate (BaTiO3).
Ceramic dielectrics are widely used in high-frequency and high-voltage applications, such as capacitors, resonators, and transducers. They are also used in the fabrication of multilayer ceramic capacitors (MLCCs), which are essential components in modern electronic devices.
One of the main advantages of ceramic dielectrics is their ability to be engineered to have specific properties by controlling their composition and microstructure. For example, the dielectric constant of barium titanate can be tuned by doping with other elements or by controlling the grain size and porosity of the material.
However, ceramic dielectrics also have some limitations. They are typically brittle and have a low mechanical strength, which can make them difficult to process and handle. They also have a relatively high manufacturing cost compared to other types of dielectric materials.
Polymer Dielectrics
Polymer dielectrics are organic materials that are composed of long chains of repeating units called monomers. They are known for their low dielectric constant, high dielectric strength, and excellent flexibility and processability. Some common examples of polymer dielectrics include polyethylene, polypropylene, and Teflon (polytetrafluoroethylene).
Polymer dielectrics are widely used in low-frequency and low-voltage applications, such as insulation for cables and wires, capacitor dielectrics, and printed circuit boards. They are also used in the fabrication of flexible electronics and wearable devices.
One of the main advantages of polymer dielectrics is their low cost and ease of processing. They can be easily molded, extruded, or printed into various shapes and sizes, making them suitable for large-scale manufacturing. They also have a high resistance to moisture and chemicals, which makes them suitable for use in harsh environments.
However, polymer dielectrics also have some limitations. They typically have a lower dielectric constant and higher dielectric loss compared to ceramic dielectrics, which can limit their use in high-frequency applications. They also have a lower thermal stability and can degrade at high temperatures.
Glass Dielectrics
Glass dielectrics are amorphous materials that are composed of silicon dioxide (SiO2) and other metal oxides. They are known for their high dielectric strength, low dielectric loss, and excellent thermal and chemical stability. Some common examples of glass dielectrics include fused silica, borosilicate glass, and soda-lime glass.
Glass dielectrics are widely used in high-voltage and high-temperature applications, such as insulators for power transmission lines, substrates for electronic devices, and windows for microwave ovens. They are also used in the fabrication of optical fibers and photonic devices.
One of the main advantages of glass dielectrics is their excellent insulating properties and high resistance to electrical breakdown. They also have a low coefficient of thermal expansion, which makes them suitable for use in applications that require dimensional stability.
However, glass dielectrics also have some limitations. They are typically brittle and have a low mechanical strength, which can make them difficult to process and handle. They also have a relatively high manufacturing cost compared to other types of dielectric materials.
Composite Dielectrics
Composite dielectrics are materials that are composed of two or more distinct phases, such as a polymer matrix reinforced with ceramic or metal particles. They are designed to combine the
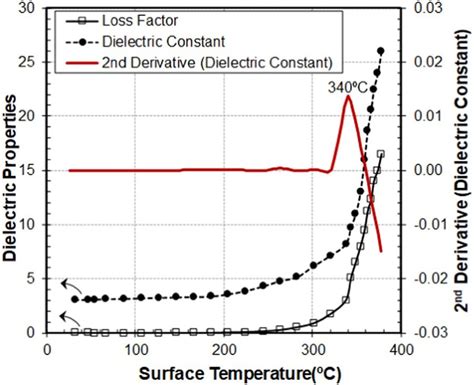
Leave a Reply